Cellular, Hormonal, and Behavioral Responses of the Holothuroid Cucumaria frondosa to Environmental Stressors
- 1Department of Ocean Sciences, Memorial University, St. John’s, NL, Canada
- 2Society for the Exploration and Valuing of the Environment, St. Philips, NL, Canada
Holothuroids (sea cucumbers) are one of the most ubiquitous groups of benthic animals found across diverse marine ecosystems. As echinoderms, they also occupy an important place in the evolutionary hierarchy, sitting close to vertebrates in the deuterostome clade, making them valuable multidisciplinary model organisms. Apart from being ecologically and phylogenetically important, many species are commercially exploited for luxury seafood markets. With the global rise of aquaculture and fisheries, management and protection of these valuable species relies on a better understanding of how their immune systems respond to environmental and anthropogenic stressors. Here, the cellular, hormonal and behavioral indicators of stress in the North Atlantic sea cucumber Cucumaria frondosa were examined. The immediate and carry-over (post recovery) effects of a 1-hour exposure to low salinities or to emersion (at two temperatures) highlighted that morphoplasticity in C. frondosa was accompanied by shifts in all monitored indicators. From baseline levels measured in controls, densities of free coelomocytes increased, showing successions of specific cell types and subsequent coelomocyte aggregations, combined with a rise in cortisol levels. These responses mirrored increased fluctuations in cloacal opening rates, decreased force of attachment to the substrate, and enhanced movements and active buoyancy adjustment with increasingly severe stressors. The findings suggest that many systems of sea cucumbers are impacted by stresses that can be associated with harvesting and handling methods, with likely implications for the quality of the processed products. Gaining a deeper understanding of immune and hormonal responses of sea cucumbers is not only of broad ecological and evolutionary value, but also helpful for the development of sustainable fisheries and aquaculture practices, and conservation programs.
Introduction
Sea cucumbers (Echinodermata: Holothuroidea) are globally fished as a luxury seafood and many populations worldwide are fully or overfished (Purcell et al., 2013). Their commercial value, which can reach over 2000 USD kg–1 (Purcell, 2014) depends on the species, and a suite of visual and organoleptic properties, such as size, shape, odor, and color (Toral-Granda et al., 2008). Harvesting, holding and processing conditions all have an impact on these characteristics as well as on the nutritional quality of the final products (Yang et al., 2015; Gianasi et al., 2016; Qi et al., 2016). There is even a perceived difference in quality between wild-caught and farmed sea cucumbers, which determines their respective prices on the retail markets (Hossain et al., 2020). In addition to being economically valuable, holothuroids are an important model organism (Zhang et al., 2017) due to their position as an echinoderm in the deuterostome clade, this places them closer to vertebrates than the vast majority of other non-chordates in the evolutionary hierarchy (Smith et al., 2018).
While the anatomy of sea cucumbers appears quite simple, they have developed many unique adaptations that allow them to thrive in different marine environments across the globe. They can be suspension feeders, using branching oral tentacles to capture particulate matter from the water, or deposit feeders ingesting sedimented organic matter. They have traditionally been considered to live a fairly sedentary lifestyle apart from their free swimming larval phases (Young and Chia, 1982; Hamel and Mercier, 1996; Grantham et al., 2003), yet the recent discovery of active buoyancy adjustment (ABA) has revealed that they can travel large distances through manipulation of their water-to-flesh ratio in response to stressful situations (Hamel et al., 2019). The internal anatomy of sea cucumbers includes two main coeloms, the perivisceral cavity and the hydrovascular system. The former is the central body cavity holding most organs. The latter is comprised of the ampullae of the tube feet, the vesicle of the tentacles and the Polian vesicle, as well as numerous canals and the madreporite, which aid in a variety of processes including locomotion, feeding, and immunity (Li et al., 2013).
The two coeloms of sea cucumbers host populations of free coelomocytes (free floating in the fluid), which are considered among the most promising markers of stress (Bang, 1975; Coteur et al., 2002; de Freitas Rebelo et al., 2013; Li et al., 2013; Franchi and Ballarin, 2017; Caulier et al., 2020). Moreover, coelomocytes play pivotal roles in immune functions; they have been described across different classes of echinoderms as the first line of immune defense against foreign particles/cells, including in Asteroidea (sea stars; Smith and Davidson, 1992, 1994); Echinoidea (sea urchins; Pinsino et al., 2007; Brothers et al., 2016) and Holothuroidea (sea cucumbers; Ramírez-Gómez et al., 2010; Galimany et al., 2018). Among Holothuroidea, coelomocytes have consistently been classified as phagocytes, morula cells, hemocytes, fusiform cells, and crystal cells (Caulier et al., 2020). They are known to play roles in recognition of non-self-materials, cytotoxic defense, fluid circulation, clotting, and encapsulation (Canicatti et al., 1989; Chia and Xing, 1996; Smith et al., 2018). Phagocytes are the most prominent coelomocytes and they are documented to undergo transformation from petaloid to filopodial morphologies (Edds, 1980; Chia and Xing, 1996; Smith et al., 2018). Coelomocytes that were monitored in sea cucumbers undergoing physical harm, intense disturbance/relocation and illness were shown to increase in abundance as both free and aggregated forms (Gross et al., 1999; Hou et al., 2019; Caulier et al., 2020) a phenomenon also recorded in sea urchins (Ridder and Jangoux, 1984; D’Andrea-Winslow et al., 2012; Majeske et al., 2013; Branco et al., 2014; Chiaramonte et al., 2019).
The aggregation of free coelomocytes in echinoderms has been superficially mentioned in the literature but its drivers and roles have long remained poorly understood (Ridder and Jangoux, 1984; Canicatti and Seymour, 1991; Jans et al., 1995). In addition, aggregates are known under different terms in echinoderms, including encapsulates, bodies, aggregates or syncytia (Dan-Sohkawa et al., 1995a,b; Söderhäll, 2010), and as nodules in insects (Satyavathi et al., 2014). In Holothuroidea, they were historically described as brown bodies (Hetzel, 1965; Ridder and Jangoux, 1984; Canicatti and Quaglia, 1991) despite the various colors that characterize them, making aggregates a more accurate designation. Caulier et al. (2020) provided a detailed study of coelomocytes in the sea cucumber Cucumaria frondosa that included the formation of aggregates and their transition from un-pigmented to red and brown variants. The study also showed that their abundance rose with increasing severity of applied stressors, including exposure to a predator and injury.
Along with cellular markers, cortisol is a well-established hormonal marker of stress in vertebrate model systems (Xu et al., 2019; Sandner et al., 2020; Uren Webster et al., 2020). Only recently have researchers begun testing cortisol levels in sea cucumbers (Pei et al., 2012; Chen et al., 2018a; Hou et al., 2019) and other non-vertebrate taxa like mussels (Chen et al., 2018a; Binder et al., 2019). Cortisol levels in the sea cucumber Apostichopus japonicus rose from 4 mmol L–1 to above 6 mmol L–1 when individuals were placed in situations known to cause stress or agitation, e.g., high conspecific density, emersion or starvation (Pei et al., 2012; Xia et al., 2017; Hou et al., 2019).
The sea cucumber Cucumaria frondosa is common and abundant in North Atlantic and Arctic waters (Gianasi et al., 2020). It is also one of the most important emerging commercial species in the North Atlantic and is being considered a promising candidate for multitrophic aquaculture (Nelson et al., 2012; Sun et al., 2020). Over the years, behavioral responses of C. frondosa have been studied and correlated with their well-being (Gianasi et al., 2020). Among them, the rhythm of cloacal opening provides a metric to evaluate respiration rates, which were demonstrated to increase when individuals were exposed to various stressors (Gianasi et al., 2015; Ammendolia et al., 2018). The force of attachment of the ambulacral podia to the substrate was also used (Hamel et al., 2019). Detachment from the substrate combined with increased motility through active buoyancy adjustments have been triggered by high conspecific densities, encounters with predators, sudden decreases in salinity, and increased turbidity (Sun et al., 2018; Hamel et al., 2019).
The present study took an integrative approach, seeking to explore the link between behavioral and internal biomarkers of health and stress in the sea cucumber Cucumaria frondosa. The objective was to tease out the relationship between free coelomocyte abundance and the specificity, type and abundance of their aggregates, cortisol levels in the fluid of the hydrovascular system, and known behaviors. The various components of immune defense were examined in individuals exposed to stressors selected to mimic situations commonly experienced by sea cucumbers as they are harvested (e.g., exposure to air, temperature shocks, and salinity changes) as stated in Gianasi et al. (2016). Exploring the link between cellular and hormonal responses could help devise more reliable means of monitoring, quantifying, and comparing the stress responses of sea cucumbers with a dual aim to help mitigate their impacts on the commercial products and provide a framework for conservation and evolutionary studies. The main hypothesis was that an increase in free coelomocytes and aggregates would be proportional to the severity of the stressors and would follow a rise in cortisol levels in the hydrovascular system.
Materials and Methods
Collection and Holding Conditions
Individuals of Cucumaria frondosa were collected in the subtidal zone (10.5-12 m depth) of Tors Cove, Newfoundland and Labrador (47.2172°N, 52.8515°W) during the fall of 2019. To minimize stress during transport and holding, all individuals were hand collected by divers and transported at low densities inside large coolers filled with seawater. Special measures were taken to ensure that individuals were handled gently and never exposed to air at any time throughout their relocation. Individuals were distributed in a 500 L tank supplied with unfiltered, running ambient seawater at a rate of 250 L h–1. Sea cucumbers were held in these conditions for a minimum acclimation period of 2 weeks prior to experimental use. The water temperature in the holding tank fluctuated naturally over the annual cycle between 0 and 8°C, at a salinity around 35 psu, and a natural photoperiod with peak light intensity of ≤200 lux (measured using Traceable® Dual Display Light Meter) was provided through large windows. All individuals fed on natural seston present in the ambient unfiltered seawater. Only healthy individuals of medium size (13.5 ± 2.2 cm SD contracted length) that were firmly attached to the substrate, with tentacles periodically extended and showing no sign of injuries, were used in the experiments.
Experiments were conducted in clear bare tanks of 20 L (267 × 394 × 216 mm), using a single sea cucumber per tank. Both control and exposure tanks were randomly distributed in shelves, and all were lined with white corrugated plastic along the bottom to enhance contrast between the background and the brown sea cucumbers for time-lapse photography. Illumination provided by fluorescent lights covered in a mesh shade was adjusted to 200 lux, as per Gianasi et al. (2015). Black tarps were used to isolate the tanks from other light sources. Where applicable, the flow rate in the tanks was set to 42 L h–1. Sea cucumbers were always moved from holding to experimental tanks inside 1-2 L large beakers filled with seawater to keep them submerged at all times; surgical gloves were used as needed to avoid touching them directly. The exposure treatments began directly following relocation.
To assess both the acute and carry-over effects of stressors, five control and five exposed individuals for each treatment group were processed at two points, the first was immediately after 1 h exposure to the stressor (described below) and the second was following 1 h of exposure to the stressor plus a recovery period of 23 h under control conditions (similar to holding conditions) totaling a 24 h treatment. After the exposure and recovery (after 1 h and 24 h, respectively), all individuals were first photographed and their whole-body wet weight (after draining for 3 min on paper towel), mid-length circumference and contracted length were recorded.
Treatments
Air Exposure Treatments
Two air temperatures were tested using bare tanks: 17 and 5°C. The higher setting (16.8 ± 0.6°C) is typically experienced by sea cucumbers at capture and during offloading in summer; and was achieved by keeping the tanks at room temperature. The lower setting (5.2 ± 0.8°C) is experienced by sea cucumbers stored in ship hauls and refrigerated trucks during transport to the plants, as per Gianasi et al. (2016). It was achieved by placing the experimental tank into a larger 40-L vessel filled with crushed ice (Supplementary Figure 1). During both experiments, the temperature was recorded using a digital thermometer (Zacro®, Model FBA_ZDT1-AUX-1). To minimize desiccation of sea cucumber epithelia and reduce air movements, a lid was used to seal and keep the humidity inside the bare tanks at ∼91%, measured with a hygrometer/thermometer (Thomas scientific Traceable®). The controls for each of the two air-exposure treatments consisted of five individuals transferred to separate seawater-filled tanks under environmental conditions similar to holding tanks (described above; mean of 7.3°C).
Salinity Exposure Treatments
Two salinities commonly experienced by sea cucumbers during transport post-harvesting were tested (15 and 22 psu) and compared to ambient salinity typical off the coast of Newfoundland (control, 35 psu). To reduce the salinity, natural seawater at 35 psu was mixed with filtered, demineralized freshwater until the desired level (measured with a Milwaukee MA871 Refractometer) was reached (Supplementary Figure 1). As salinity experiments were conducted under static conditions, dissolved oxygen (O2) was measured periodically (OaktonTM DO Six + Meter) to ensure its levels remained optimal and comparable to flow-through conditions for the duration of the exposure period. Under salinities of 35, 15 and 22 psu, the dissolved oxygen levels were 104.2 ± 8.6% SD, 91.7 ± 8.5% SD, and 98.3 ± 12.1% SD, respectively, i.e., in the range of normoxia and well above hypoxia (Suh et al., 2014; Huo et al., 2018, 2019).
Biomarker Analyses
Cellular Markers (Free and Aggregated Coelomocytes)
The body wall of each sea cucumber was opened longitudinally from anus to mouth between two rows of tube feet using scissors or a scalpel, keeping the incision shallow to avoid puncturing the hydrovascular system and allow the removal of an intact Polian vesicle (PV). While drawing fluid across the body wall using a syringe has commonly been used (e.g., Fontaine and Lambert, 1977; Galimany et al., 2018; Hou et al., 2019), this blind technique does not guarantee that only coelomic fluid is sampled because the respiratory tree, intestine, gonad, and hydrovascular system can be accidentally punctured during the process. On the other hand, the PV has not only been shown to provide a suitable source of coelomocytes and their aggregates for quantitative assessments (Li et al., 2019; Caulier et al., 2020; Hamel et al., 2021), but it has two major advantages: (i) it ensures the collection of fluid holding uncontaminated coelomocytes and aggregates, and (ii) it standardizes the origin and volume of samples across individuals, for increased reproducibility. The whole PV was emptied into a 25 mL Falcon tube to record fluid volume. To determine the number and type of coelomocytes, the fluid was resuspended using a mini vortexer (MV 1 from IKATM) for 3 s and 10 μl was loaded in an hemocytometer (Neubauer, LW Scientific). Contrary to conventional protocol, the coverslip was placed on the chamber after (rather than before) it was loaded to make sure naturally formed coelomocyte aggregates would enter the chamber. Because clotting is a main issue when working with coelomocytes (Smith et al., 2018; Caulier et al., 2020), the samples had to be analyzed immediately after sampling (within 5 min). It should also be noted that the use of anticoagulants (like ethylenediamine tetraacetic acid) was explored as a possible solution to coelomocyte clotting post extraction. However, anticoagulants can cause pre-existing coelomocyte aggregates to break down, creating bias in the results. Free coelomocytes (individual cells) and aggregates (coelomocytes found in groups or clumps) were measured (Feret diameter; i.e., the longest possible diameter) and photographed under a light microscope (Nikon Eclipse 80i) coupled to a digital camera (Olympus DP73). Identification of free coelomocyte types was based on Caulier et al. (2020) for Cucumaria frondosa, complemented by studies of other holothuroids (Chia and Xing, 1996; Smith et al., 2018). Phagocytes were subdivided in two categories, i.e., inactive (pseudopodial fans still wrapped around the nucleus also known as the bladder form; Supplementary Figure 2A), and active (microtubule fans extended as petaloid or filapodial forms; Supplementary Figures 2B,C; Kindred, 1924). The presence of morula cells, fusiform cells, and crystal cells was also assessed (Supplementary Figures 2D–H).
The coelomocyte aggregates were divided in two classes: small and large, which corresponded nearly perfectly with early and mature forms, respectively, based on the classification proposed by Caulier et al. (2020). The small aggregates were characterized by a diameter <200 μm and mostly composed of translucent coelomocytes (with minimum size of ∼5 μm in diameter). These small aggregates were counted using a hemocytometer (method described above). Large aggregates were composed of coelomocytes grouped in reddish clumps measuring ≥200 μm in diameter (maximum size of 6600 μm in Feret diameter). Because these aggregates were too large to be analyzed with the hematocytometer, a 2 mL subsample of PV fluid was diluted with 10 mL of filtered seawater and poured into a gridded Petri dish (square, 36 grids, 10000 mm2). Large aggregates were counted in five grid sections selected by a random number generator (CalculatorSoup©).
Hormonal Marker (Cortisol)
Two subsamples of fluid (1 mL) from the extracted PV fluid were transfer into separate Eppendorf vials and stored at −80°C within 10 min of extraction, to be used for cortisol analysis. The frozen subsamples were thawed, and their pH lowered to 1.5 – 2.0 using 0.5 M HC1 before washing once with 4 mL of undiluted methylene chloride, following standard procedure for a competitive cortisol ELISA assay (Cayman Chemical – Item 500360). To wash, methylene chloride was added to the fluid sample and vortexed for 5 s. After being allowed several minutes to separate, the clear bottom layer of methylene chloride was removed, and the remainder was evaporated under a nitrogen stream before adding 250 μL of ELISA buffer. Preparation of assay-specific reagents followed the ELISA kit protocol (Cayman, Item No. 500360). Before plating, each sample of extracted cortisol was centrifuged at 4000 rpm for 5 min. A 96-well plate was used to run all reagents and samples in duplicate (e.g., experimental samples, the 9-point standard curve, blank, total activity, non-specific binding, and maximum binding wells). Following standard protocol, the plate was left to incubate for 24 h, washed and then shaken on an orbital shaker for 90 min. The plate was then shaken mechanically for 3 s on the microplate reader (Molecular Devices SpectraMax® M5) and read using a wavelength of 420 nm and the SoftMax® Pro v7.1 software. Data were analyzed using an Excel program designed by Cayman Chemical for this ELISA kit and publicly available (ELISADouble1). Any readings outside the standard curve were removed as per Binder et al. (2019).
Behavioral Markers
Force of Attachment and Cloacal Opening
The force of attachment of the sea cucumbers to the substrate was quantified by attaching a zip tie to the mid-circumference of the body and pulling perpendicularly with a spring balance (Ohaus®, Model 8008-MO) as per Hamel et al. (2019). The weight necessary to detach the individual was converted to force in Newtons (1 N = 101.9716 g). To quantify cloacal opening rhythm in Cucumaria frondosa, based on the work of Gianasi et al. (2015), the frequency of opening/closing of the anus (inspiration/expiration) was visually assessed for seven min in triplicates.
Behavioral Scores
All other behavioural activity levels measured over the recovery period (Supplementary Table 1) were monitored using time-lapse videography. Two cameras were used (Brinno TLC 200 Pro and Brinno MAC 200 DN) combined with infrared lighting (ICAMI IR Illuminators, 96 pcs), which allowed continuous recording (night and day). They were mounted above the tanks to capture the entire experimental arena and set to take one picture every 10 s, which were automatically stitched into clips by the camera software. Each metric (i.e., movement and speed, degree of attachment to the substrate, body inflation) was assigned a cumulative score on a scale from 0–4, with 0 indicating baseline levels (normal shape, immobile, firmly attached to the substrate with tentacles either extended or retracted) and 4 indicating extreme behavior [ABA or full inflation of the body cavity, tentacle retraction combined with complete detachment from the substrate, as per Hamel et al. (2019)]. Intermediate scores (0.5–3.5) reflected the extent to which one or more parameters were affected, including body contractions, locomotion or rolling, bloating of the body wall with or without ambulacral podia extended (details in Supplementary Table 1).
Data Analysis
Two-way analysis of variance (ANOVA) was conducted for each treatment (17, 5°C, 15, 22 psu) to compare the cellular and hormonal data over both the exposure (exposed vs. control) and time (1 h vs. 24 h). All assumptions for parametric tests were met and any metrics of significance were investigated using pairwise comparison (Holm-Sidak method). Any extreme outliers were removed within each category provided that their removal did not change any overarching trends. The results from control individuals in the cortisol treatment group were not significantly different and were pooled together. All tests were performed with SigmaPlot statistical software and evaluated using α = 0.05 to indicate strong significance, although p-values < 0.1 were noted as potential indicators of moderate significance based on Fisher’s sorting method (Fisher, 1934). This approach is based on calls from statisticians to move away from arbitrary measures of significance (Yoccoz, 1991; Wasserstein and Lazar, 2016; Dushoff et al., 2019; Wasserstein et al., 2019).
To calculate percent increase in cell densities relative to baseline, the difference between the initial and final density in each exposed individual was divided by the mean baseline (control) cellular density, multiplied by 100 and averaged (mean ± SD). For comparisons of cell densities across time points, the difference between the means of each group were compared as a percentage.
Results
Cellular Markers
Free Coelomocytes
Individuals exposed to stressors, globally displayed higher densities of free coelomocytes in the PV fluid than their respective controls (Figure 1). Specifically, an increase in coelomocyte density occurred after 1 h in three of the four treatments (17°C air, 15 and 22 psu salinities, Figures 1A,C,D) while it occurred only after 24 h under the 5°C air treatment (Figure 1B; for statistics see Supplementary Table 2). All treatments except 22 psu generated a greater departure from baseline coelomocyte densities after the recovery period (24 h) than immediately after exposure (1 h) (Figure 1 and Supplementary Table 3).
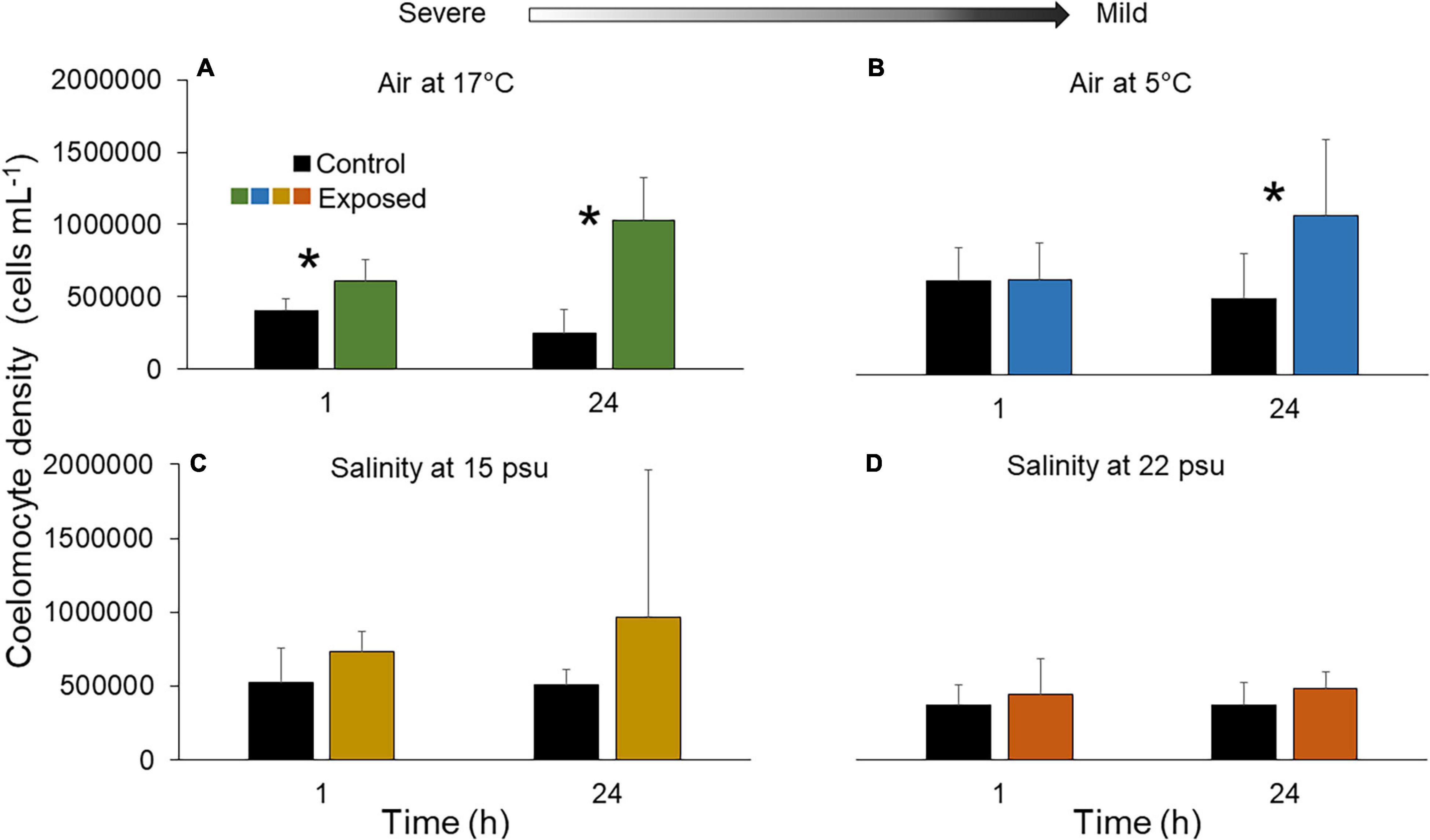
Figure 1. Density of free coelomocytes in fluid of the Polian vesicle (mean ± SD, n = 5–10) after exposure of sea cucumbers to (A) 17°C air (B) 5°C air (C) 15 psu salinity and (D) 22 psu salinity. The asterisk (*) indicates significant differences between control and exposed individuals (p < 0.05) at each time point. There is no statistical difference between treatment groups at different time points (p > 0.05). Arrow indicates severe and mild stressors relative to the control. Measurements were made immediately after the exposure (1 h) and after a 23 h recovery period (total of 24 h). Main statistical results are shown in Supplementary Table 2.
Analysis of coelomocyte types showed that the most abundant were the phagocytes in all control and treatment groups (Figure 2). They represented 81.3 ± 8.8% of free coelomocytes after 1 h and 92.0 ± 2.8% after 24 h under 17°C air exposure (Figure 2A). Similar proportions were also seen under 5°C air, and both salinities (Figures 2B–D). In comparisons with baseline levels, phagocyte densities under 17°C air exposure were higher after both 1 h and 24 h, with peak increase occurring in the latter (216.7 ± 84.3%; F1,23 = 4.45, p = 0.046; Figure 2A). In air at 5°C, phagocyte densities showed no departure from baseline after 1 h (Figure 2B) and were 88.0 ± 75.4% higher after 24 h; this increase was too variable to be supported statistically (F1,25 = 1.15, p = 0.30; Figure 2B). When sea cucumbers were exposed to 15 or 22 psu salinity, phagocyte densities showed no clear departure from baseline (Figures 2C,D).
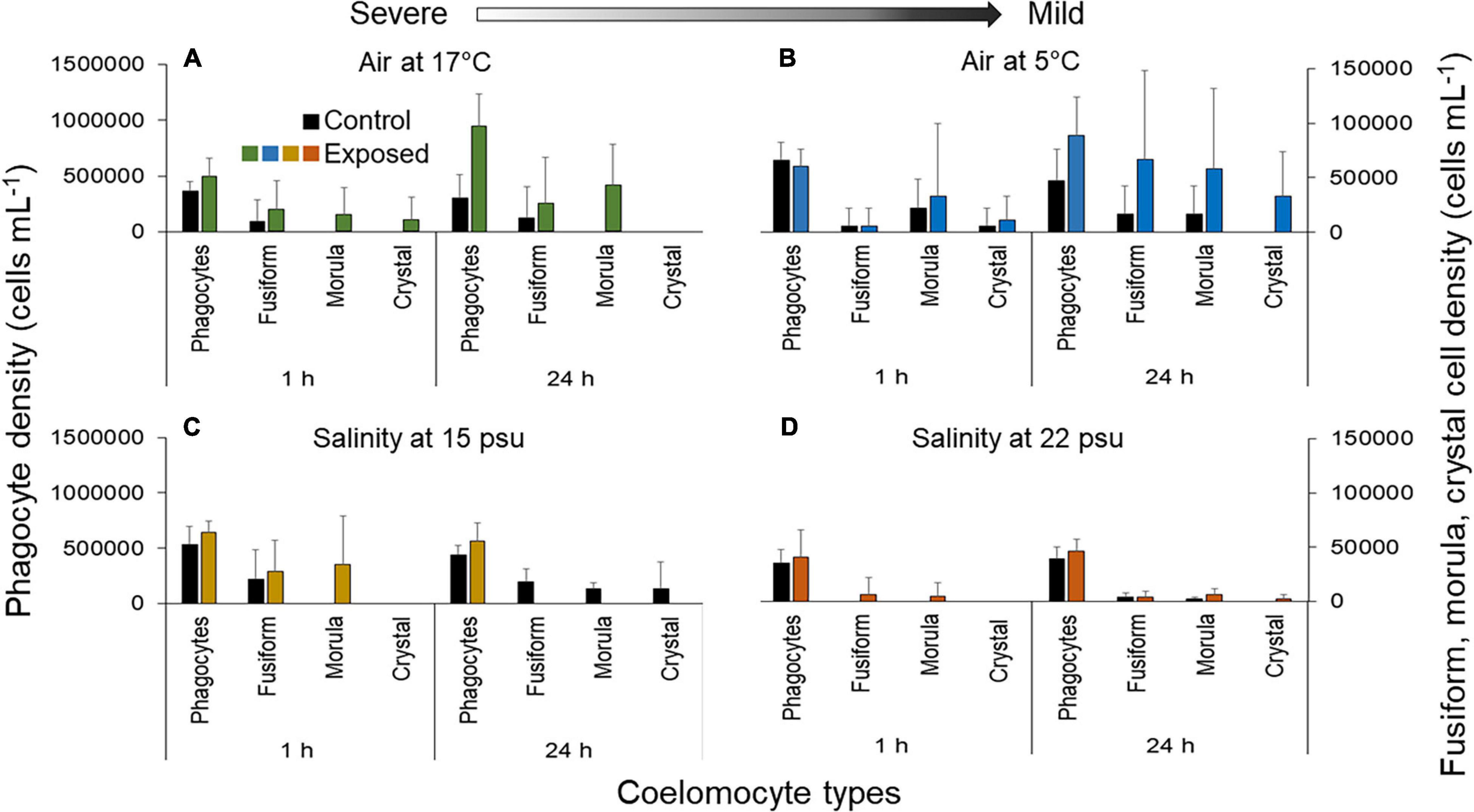
Figure 2. Number of free coelomocytes (separated by cell type) per mL of fluid in the Polian vesicle fluid of sea cucumbers (mean ± SD, n = 5–10) following exposure to (A) 17°C air, (B) 5°C air, (C) 15 psu salinity, and (D) 22 psu salinity. Values on the left axis correspond to the density of phagocytes and values on the right axis correspond to the density of fusiform, morula and crystal cells (note the order of magnitude difference in scales). Measurements were made immediately after the exposure (1 h) and after a 23 h recovery period (total duration of 24 h). Arrow indicates severe and mild stressors relative to the control.
Looking at other coelomocyte types, when sea cucumbers were exposed to 17°C air, the density of fusiform cells showed a variable increase of 140.0 ± 309.8% after 1 h and 114.3 ± 327.3% after 24 h (F1,23 = 1.05, p = 0.32; Figure 2A). Both morula and crystal cells, which were absent from the controls, appeared in low numbers after 1 h (Figure 2A). Despite the fact that the density of morula cells remained higher than baseline after 24 h, the crystal cells disappeared (Figure 2A). After 1 h under 5°C air, the fusiform cell densities remained comparable to baseline, but densities of morula and crystal cells were higher by 50 ± 41.7% and 100.0 ± 122.2%, respectively, (F1,25 = 0.71, p = 0.41; F1,25 = 4.66, p = 0.040; Figure 2B). When exposed to 15 psu salinity, the fusiform cells increased after 1 h, the morula cells (absent in the controls) became detectable (35,000 ± 19,512 cells; F1,16 = 0.48, p = 0.50; t = 2.67, p = 0.0.016, respectively) and no crystal cells were recorded after 1 h. After 24 h, the fusiform and morula cells disappeared (Figure 2C). At 22 psu, the morula cells increased compared to controls after 1 h (F1,20 = 2.96, p = 0.10; Figure 2D). However, the fusiform cells decreased by 49.4 ± 133.9% relative to baseline and no crystal cells were noted (Figure 2D). After 24 h, the fusiform cells were slightly higher than baseline, morula cells showed an increase of 188.0 ± 245.6% (F1,20 = 0.0030, p = 0.96; F1,20 = 1.51, p = 0.25), and the crystal cells appeared for the first time in low numbers (∼2000 cells).
Phagocytes were further subdivided into inactive and active cells. After 1 h under 17°C air exposure, the inactive forms represented roughly half that of exposed individuals (32.6 ± 20.8%; F1,23 = 3.86, p = 0.061; Figure 3A). After 24 h, proportions were similar in controls and exposed individuals (Figure 3A). An increase in inactive phagocytes was observed under 17 and 5°C air after 1 h, at 15 psu salinity after both 1 and 24 h and at 22 psu after 24 h only (Figures 3B,C). Inverse trends were noticed under 5°C air exposure after 24 h and at 22 psu salinity after 1 h, whereby the percentage of inactive phagocytes in exposed individuals compared to controls decreased although not significantly from 53.2 ± 27.6% to 36.3 ± 26.1% and 57.1 ± 19.6 to 43.8 ± 37.7%, respectively (t = 0.11, p = 0.92; t = 1.64, p = 0.12; Figures 3B–D).
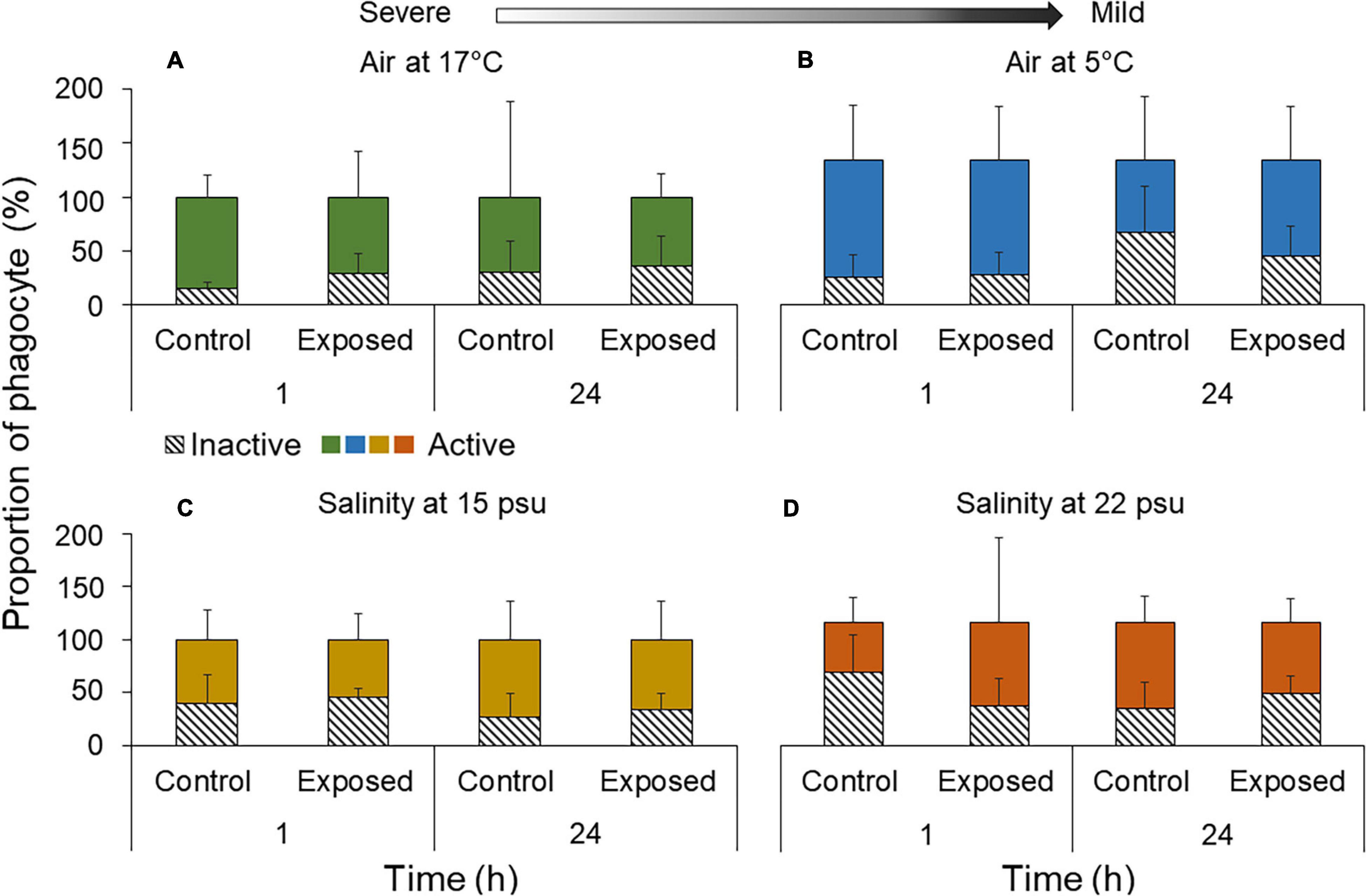
Figure 3. Proportion of phagocytes (active vs. inactive) per mL of Polian vesicle fluid (mean ± SD, n = 5–10) following exposure to (A) 17°C air, (B) 5°C air, (C) 15 psu salinity, and (D) 22 psu salinity. Measurements were made immediately after the exposure (1 h) and after a 23 h recovery period (total duration of 24 h). Arrow indicates severe and mild stressors relative to the control.
Small Coelomocyte Aggregates
In groups exposed to 17°C air, the density of small (early stage) aggregates increased by 38.3 ± 45.6% after 1 h (t = 2.73, p = 0.012) and returned to baseline values after 24 h (Figure 4A and Supplementary Table 2). Inversely, under 5°C air exposure, small aggregate densities were similar between control and treatment groups after 1 h but were 91.5 ± 81.9% higher in exposed individuals after 24 h (F1,16 = 3.86, p = 0.061; Figure 4B). In individuals exposed to 15 psu salinity, the small aggregates increased compared to controls after 1 h (F1,16 = 0.070, p = 0.80) and fell to control levels after 24 h. The trend was inversed at 22 psu, where densities of small aggregates in individuals hovered around baseline after 1 h, and the difference amplified to 196.4 ± 59.9% after 24 h (t = 2.39, p = 0.028; Supplementary Table 2 and Figure 4D).
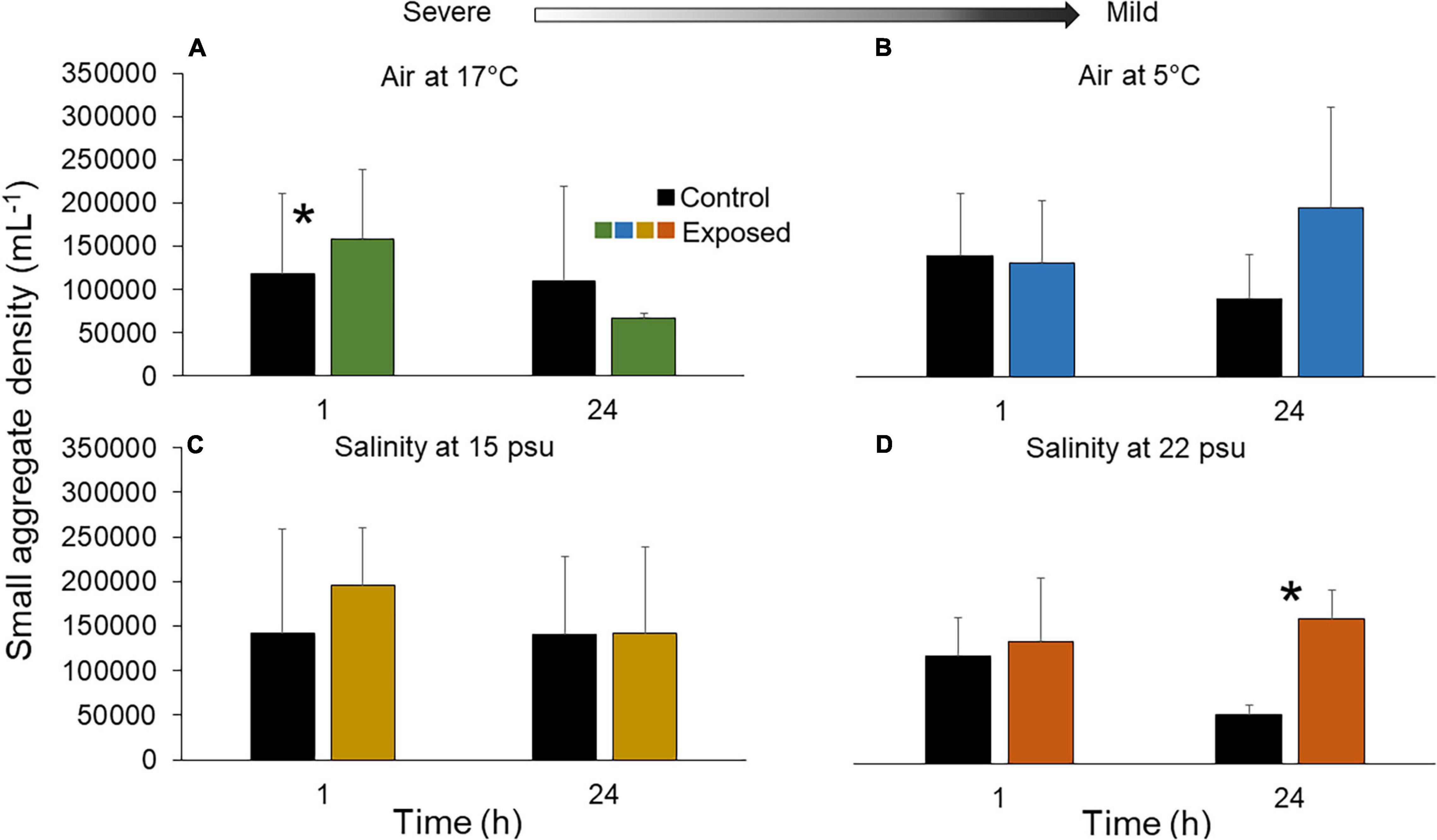
Figure 4. Density of small aggregates in fluid of the Polian vesicle (mean ± SD, n = 5–10) after exposure of sea cucumbers to (A) 17°C air (B) 5°C air (C) 15 psu salinity and (D) 22 psu salinity. The asterisk (*) indicates significant differences between control and exposed individuals (p < 0.05) at each time point. There was no statistical difference between treatment groups at different time points (p > 0.05). Arrow indicates severe and mild stressors relative to the control. Measurements were made immediately after the exposure (1 h) and after a 23 h recovery period (total of 24 h). Main statistical results are shown in Supplementary Table 2.
Large Coelomocyte Aggregates
Densities of large (mature stage) aggregates were overall quite variable. Under 17°C air exposure, the density fluctuated around baseline after both 1 and 24 h (Figure 5A and Supplementary Table 2). Under 5°C air, no clear departure occurred after 1 h but densities were 159.6 ± 411.0% higher after 24 h (Figure 5B; t = 1.7, p = 0.10). In the salinity treatments, individuals exposed to 15 psu displayed an increase in large aggregates by 500.0 ± 300.0% after 24 h only (F1,12 = 0.049, p = 0.83; Figure 5C), whereas individuals exposed to 22 psu showed elevated densities both after 1 h exposure and 24 h recovery, by 33.3 ± 103.3% and 100.0 ± 282.8%, respectively (Figure 5D).
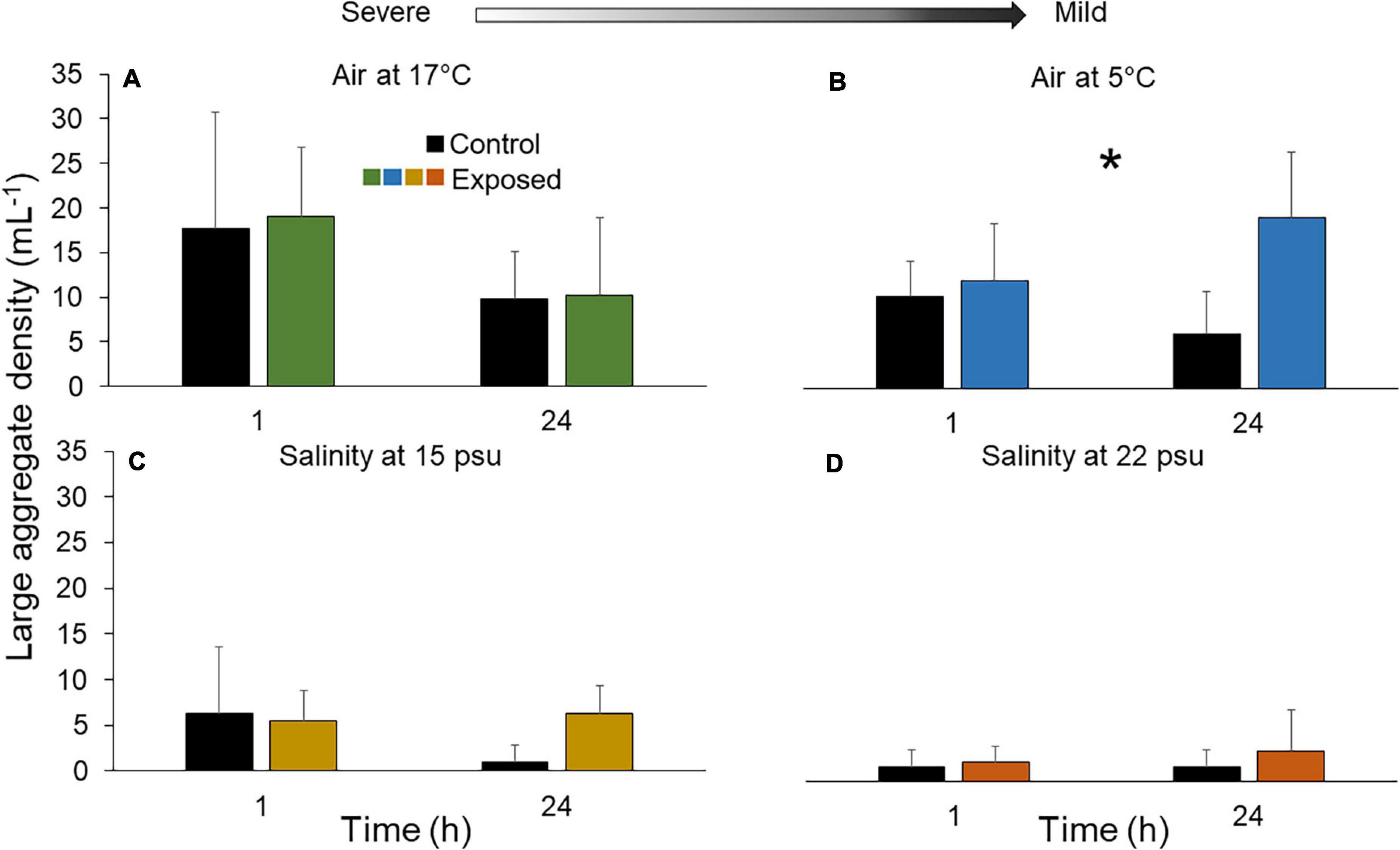
Figure 5. Density of large aggregates in fluid of the Polian vesicle (mean ± SD, n = 5–10) after exposure of sea cucumbers to (A) 17°C air (B) 5°C air (C) 15 psu salinity and (D) 22 psu salinity. The asterisk (*) indicates significant differences within one treatment group (p < 0.05), however, pairwise comparisons showed no statistical significance between control and exposed individuals at either time point (1 and 24 h). There was no statistical difference between treatment groups at different time points (p > 0.05). Arrow indicates severe and mild stressors relative to the control. Measurements were made immediately after the exposure (1 h) and after a 23 h recovery period (total of 24 h). Main statistical results are shown in Supplementary Table 2.
Hormonal Marker
Overall, cortisol levels in the PV were variable but increased in all treatments after 1 h, except exposure to air at 5°C (Figure 6 and Supplementary Table 2). This increase was statistically significant when individuals were exposed to 17°C air, passing from a mean of 25.2 pg mL–1 in controls to 112.0 pg mL–1 under the stressor (an increase of ∼345%; Figure 6A). Values returned to baseline after 24 h (Figure 6A). Under 5°C air, no cortisol increase was noted at any time point (Figure 6B). In individuals exposed to 15 psu, mean cortisol level was 21.6 pg mL–1 under control and 33.0 pg mL–1 under the stressor (representing an increase of ∼64%); values remained elevated until the end of the experiment (Figure 6C). In individuals exposed to 22 psu salinity, the mean cortisol level after 1 h showed an increased from 14.6 pg mL–1 in the control to 54.9 pg mL–1 under the stressor (∼276%; Figure 6D). Values remained higher than in controls until the end of the experiment (Figure 6D).
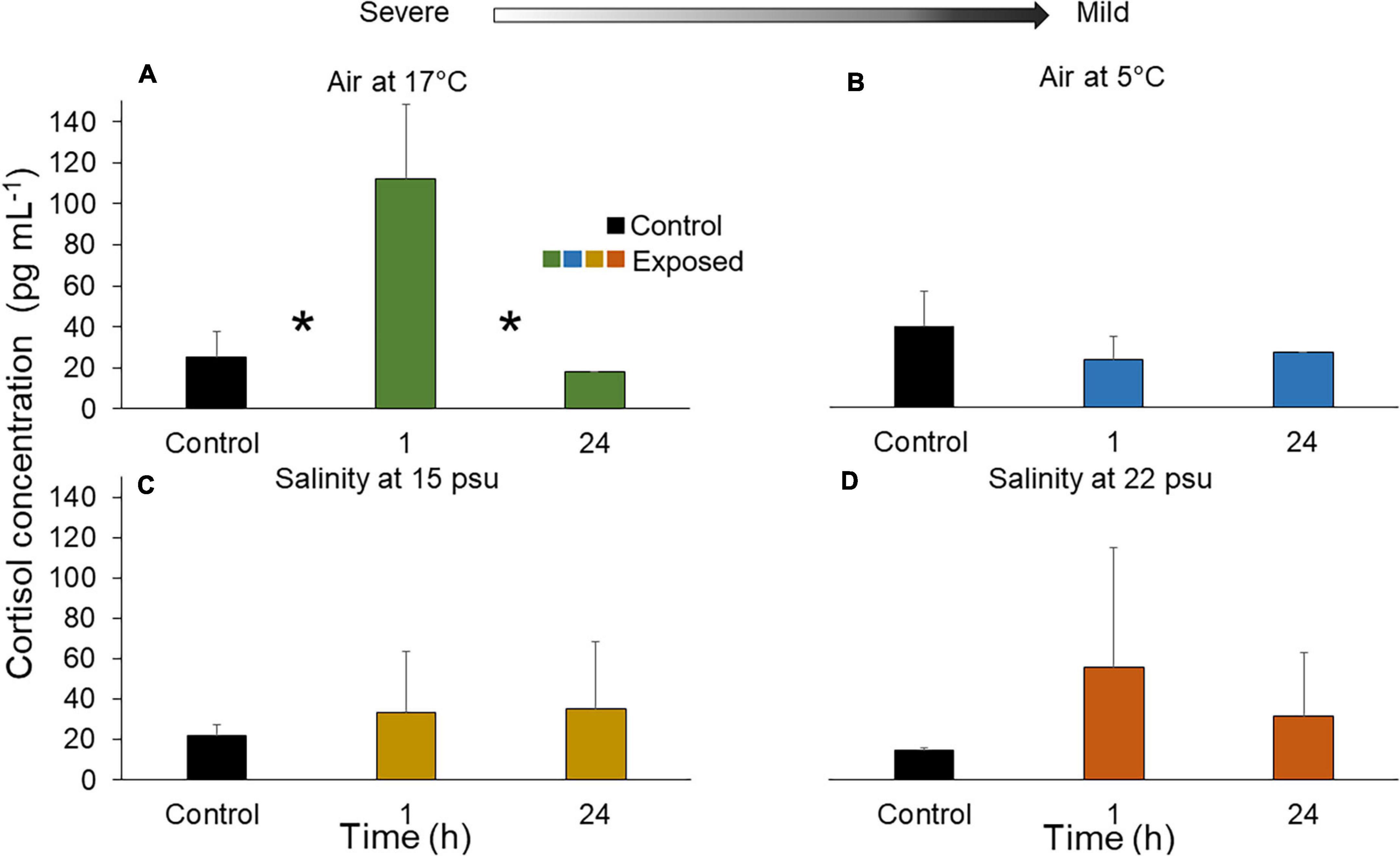
Figure 6. Cortisol concentration (pg mL– 1) after exposure of sea cucumbers to (A) 17°C air (B) 5°C air (C) 15 psu salinity and (D) 22 psu salinity. The asterisk (*) indicates significant differences within one treatment group (p < 0.05), in this case (A) the concentration between control individuals and those sampled at the 1 h mark was significantly different as well as the cortisol concentration between those sampled at 1 and 24 h. There was no statistical difference between the control and exposed individuals after 24 h (p > 0.05). Arrow indicates severe and mild stressors relative to the control. Measurements were made immediately after the exposure (1 h) and after a 23 h recovery period (total of 24 h). Main statistical results are shown in Supplementary Table 2.
Behavioral Markers
Cloacal Opening Rhythms and Force of Attachment to the Substrate
All sea cucumbers that were used for the experiments showed around 1.5 cloacal openings min–1 in holding conditions (Supplementary Figure 3). When they were exposed to air, regardless of temperature, an interruption of the cloacal movements was noted, with their anus closed most of the time (Supplementary Figure 3A). However, release of water from the respiratory tree was observed on a regular basis, with no air intake during the process in all emersed individuals. When these individuals were resubmerged in seawater for the recovery period, cloacal opening resumed and increased from 0 to 1.9 and 0 to 2.2 openings min–1after 1.5 h for 17 and 5°C treatments, respectively, (Supplementary Figure 3A). At times 2 and 3 h, individuals exposed to 17°C air exhibited cloacal opening rhythms that were 11 to 23% faster than controls (t = 5.79, p < 0.001; t = 5.46. p < 0.001). Values decreased to 2 openings min–1 after 23–24 h and were too erratic to clearly differ from control values (t = 2.27, p = 0.29; t = 2.71, p = 0.11). Under 5°C air, cloacal movements were faster than controls after 2 h at 2 openings min–1 but decreased back to control values after 2.5 and 3 h, remaining low at 1.5 opening min–1 until the end of the recovery period (t = 1.90, p = 0.69; t = 0.99, p = 0.99; t = 0.85, p = 0.98; t = 0.58, p = 0.98, respectively). Individuals exposed to salinities of either 15 or 22 psu demonstrated an immediate decrease in cloacal opening rates relative to the baseline, from 1.41 to 0.86 and 1.0 openings min–1 for 15 and 22 psu, respectively (F1,77 = 1.95, p = 0.057; t = 5.16, p < 0.001). After 1 h, the rhythm remained low at 1.0 openings min–1 under 15 psu but was higher at 1.4 openings min–1 under 22 psu (t = 3.94, p = 0.0060; Supplementary Figure 3B). From 1.5 h, individuals exposed to 22 psu exhibited baseline values until the end of the experiment (t = 1.28, p = 1.00). Under 15 psu, the average rate of cloacal opening remained low for the duration of the exposure to lowered salinity (0.8 openings min–1). After transfer to the recovery tank, cloacal openings started to increase, peaking at about 1.6 openings min–1 after 2 h. Cloacal opening rhythm returned to baseline levels by 2.5 h and remained stable until the end of the recovery (Supplementary Figure 3B).
Under both air treatments (17 and 5°C) the sea cucumbers remained unattached to the substratum over the 1 h exposure, resulting in a null force of attachment (0 N; Supplementary Figure 4). Under low salinity treatments, sea cucumbers showed a force of attachment of 0 N at 15 psu and 0.41 ± 1.00 N at 22 psu (Supplementary Figures 4C,D). After the recovery period, individuals in both air exposure treatments had returned to control values (17°C, 2.86 ± 2.53 N; 5°C 2.65 ± 1.93 N; control, 2.71 ± 1.90 N). However, individuals exposed to 15 and 22 psu salinities did not return to control levels, showing values of 1.23 ± 2.35 N and 2.53 ± 3.26 N, respectively, after 24 h, which were lower than controls (6.60 ± 8.08 N).
Behavioral Scores
Immediately after transfer to the 5 or 17°C air treatment (time 0), individuals showed increased activity scores compared to control individuals (Figure 7A). Individuals had stronger behavioral responses after 0.5 h, with scores up to 2.3 at 17°C and 1.5 at 5°C. While the scores remained high after 2.5 h under 17°C air, individuals exposed to 5°C air returned to control values after 1.5 h and remained thus until the end of the experiment. Individuals exposed to 17°C returned to baseline values after 3 h. Individuals exposed to both 5 and 17°C air showed minimum scores values of 0.5 after 23.5–24 h, similar to controls (Figure 7A).
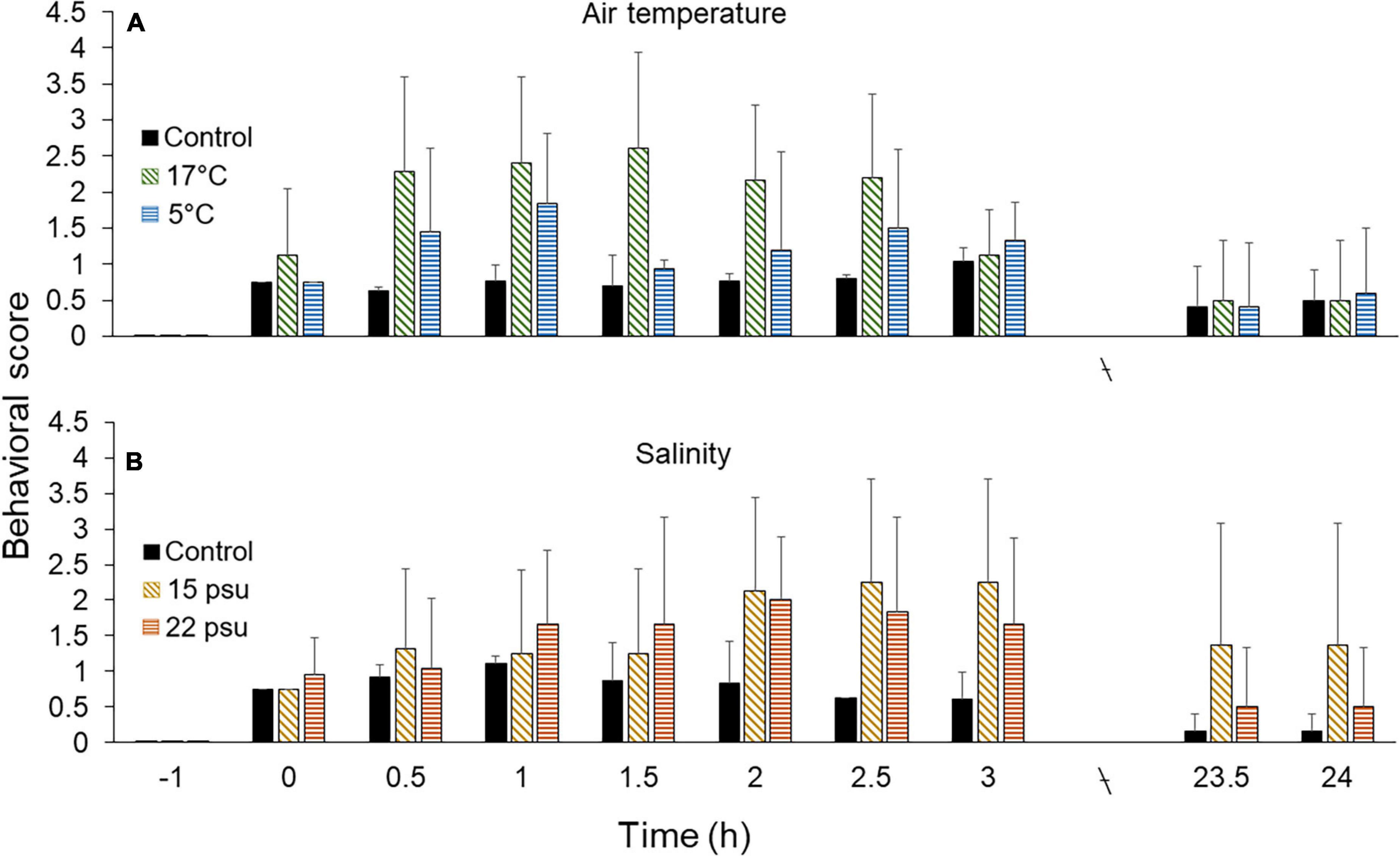
Figure 7. Behavioral intensity (0 = resting/baseline state, 4 = maximum stress) in Cucumaria frondosa at various time points (–0.5 h = negative control, 0–24 h = positive control). (A) Comparison between controls and individuals exposed to 17 and 5°C air (±SD, n = 6). (B) Comparison between controls and individuals exposed to 15 and 22 psu salinity (mean ± SD, n = 4–6).
The behavioral scores of individuals exposed to 15 psu salinity showed an increased from time 0 to a maximum after 2.5–3 h; subsequently the scores decreased slowly to values around 1.4 after 23.5–24 h, still higher than controls (Figure 7B). Individuals exposed to 22 psu salinity exhibited a sharper increase in scores over the first 1.5 h. The scores stabilized after 2 h and slightly decreased to reach 1.7 after 3 h. At the end of the recovery period (23.5 and 24 h), a few individuals still demonstrated slow movement, keeping the average scores around 1.4 for 15 psu and 0.5 for 22 psu, which were higher than controls (Figure 7B).
Discussion
Unlike most other marine species of commercial value, e.g., fishes, crabs, lobsters, shrimps, scallops, mussels, and sea urchins, sea cucumbers are not protected by any scales or hard exoskeleton that may buffer sudden environmental changes. Thus, exposure to air during natural events (e.g., washing ashore after storms) or fishing activities and exposure to salinity drops during spring thaw or live storage on ice (Gianasi et al., 2016; Hamel et al., 2019) represent acute challenges for soft-bodied sea cucumbers. In addition, sea cucumbers may undergo autolysis when they are stressed (Sun et al., 2012; Qi et al., 2016). Any deterioration of the body wall and underlying collagenous and muscle tissues, which together constitute the chief marketable products of sea cucumbers, will likely translate into commercial products of a lower grade (Purcell, 2014). In the present study, the response of the sea cucumber Cucumaria frondosa to realistic environmental stressors showed cellular, hormonal, and behavioral activity levels that related proportionally to the severity of the stressor. The greater the departure from optimal salinity and temperature conditions determined for the species (Hamel and Mercier, 1996), the stronger the response recorded, showing possible physiological and biological strategies that would confer resilience.
The results presented here are comparable to those of Wang et al. (2008) where the sea cucumber Apostichopus japonicus experienced greater challenges to its immune capacity (including phagocytic abilities and respiration) when exposed to increased water temperature than when exposed to low water temperature and lowered salinity. Here, emersion at the highest temperature and immersion at the lowest salinity elicited the greatest increase in free coelomocytes after 1 h, and subsequent spike in small aggregates, cortisol level in the coelomic fluid and the most dramatic change in cloacal opening rhythm (irrigation of respiratory tree), indicating they were most stressful for Cucumaria frondosa. These severe treatments could result in more energy expenditure to cope with tissular damages, as suspected by Gianasi et al. (2016) or other internal fluctuations through processes like the removal of dead cells. A flight reaction was shown to be elicited by the moderately low salinity (22 psu) in this species (Hamel et al., 2019) but not by the lowest salinity (15 psu), likely because it is below the tolerance threshold. Accordingly, C. frondosa was described to occur in brackish zones of the St. Lawrence Estuary (Québec, eastern Canada) but never below 22–25 psu (J-F Hamel, personal observation in Port-au-Saumon and Grande-Bergeronne).
Exposure to suboptimal conditions, even to the most severe treatments discussed above, did not generate visible damages but instead activated an arsenal of specific defenses. Lack of visible lesions is possibly due to the short exposure time, i.e., not sustained enough to completely overwhelm defense mechanisms in Cucumaria frondosa. A closer look at the various cell types involved provides some interesting insight. For instance, both exposure to air and lower salinities triggered an increase in phagocytes similar to results presented by Caulier et al. (2020) after injection of foreign particles and following trawl collection. Phagocyte counts also aligned with the cellular reaction reported by Hamel et al. (2021) who exposed C. frondosa to the predatory sea star Solaster endeca. Phagocytes were previously described as immune cells involved in phagocytosis of pathogens and in the release of humoral agents (Beck and Habicht, 1996; Rinkevich and Müller, 1996; Xue et al., 2015), suggesting that a form of internal damage occurred in sea cucumbers exposed to the most severe stressors in the present study. Fusiform cells in C. frondosa increased most markedly during emersion, as shown in A. japonicus by Xing et al. (2008), however, the function of these cells in echinoderms is still unknown (Söderhäll, 2010). Studies of fusiform cells in bivalves suggest that they aid in wound healing (Sparks, 1976), which may be occurring in the most directly exposed tissues of C. frondosa like the ambulacral podia and epithelium of the respiratory tree (both part of the hydrovascular system). Morula cells spiked during emersion under both air temperatures and immersion in low salinity, similar to a study by San Miguel-Ruiz and García-Arrarás (2007) on the sea cucumber Holothuria glaberrima who documented increasing densities of those cells in direct response to body-wall injuries. Moreover, Byrne (1986) indicated that morula cells multiplied in individuals of Eupentacta quinquesemita exposed to physical abrasion and hypothesized that these cells provide the foundation for tissue repair. Consequently, the proliferation of these cells may be triggered by many types of challenges, including in response to emersion and exposure to low salinity. Moreover, these cells reportedly secrete humoral effectors responsible for pathogen detection (Byrne, 1986; Melillo et al., 2018) suggesting that increasing density results directly from immune stress. Crystal cells in sea cucumbers were previously suggested to play a role in osmoregulation (Eliseikina and Magarlamov, 2002). Xing et al. (2008) mentioned that osmotic pressure changes triggered a reversible crystallization of the intravacuolar material in crystal cells, thereby normalizing the osmotic pressure. However, these cells did not display any detectable change or proliferation in C. frondosa during exposure to any of the low salinity treatments. In fact, the only condition where an increase in crystal cells was noticed is exposure to cold air, downplaying any role in osmoregulation, at least in C. frondosa. Importantly, holothuroids do not have integral osmoregulation mechanisms and are found strictly in marine environments (Russell, 2013). Despite this, some species like Holothuria scabra can colonize brackish areas and sustain freshwater runoff during rainy seasons, although they cope by burrowing into the sediment (Mercier et al., 1999). On the other hand, C. frondosa occurs exclusively on the surface of rocky substrata and consequently cannot burrow to withstand salinity drops. Instead, they can use active buoyancy behavior to roll or float away with the current (Hamel et al., 2019), likely to limit exposure time.
Despite the fact that phagocytes were the most common coelomocytes found in the coelomic fluid of the Polian vesicle in both control and exposed individuals of Cucumaria frondosa, these cells were not always found in their active form, which was presumed to correspond to the active form reported by Kindred (1924). Surprisingly, elevated numbers of active phagocytes were only present in individuals exposed to a salinity of 22 psu and not in the other treatments. Caulier et al. (2020) showed that the finite pool of available free phagocytes (demarginated) in the hydrovascular fluid of C. frondosa can decrease rapidly as they aggregate around foreign particles. In line with this principle, individuals exposed to the most severe stressors in the present study exhibited the lowest number of active phagocytes, suggesting that they were utilized to form aggregates, as supported by the higher number of small aggregates under those conditions. In contrast, at 22 psu, it is possible that a lower demand for tissue repair/healing was sustained by the pool of active and inactive phagocytes already available in the hydrovascular fluid.
Under most conditions tested, free coelomocytes had formed small and large aggregates immediately after the 1-h exposure. These aggregates were described as the precursor step in the expulsion of foreign particles, damaged cells, and pathogenic materials, both from the hydrovascular system and the periviceral coelom (Caulier et al., 2020). Jans et al. (1995) showed formation of “brown bodies” (i.e., aggregates in the present study) in the sea cucumber Holothuria tubulosa within 24 h of the initial immune challenge. Similarly, cell aggregations were noticed after 24 h in the sea urchin Strongylocentrotus droebachiensis (Majeske et al., 2013) and after 5 h in the sea star Asterias rubens (Gorshkov et al., 2009). In Cucumaria frondosa, their presence was noticed as early as within 1 h of exposure, suggesting that clumping occurred concurrently with the increase of free coelomocytes, i.e., almost immediately upon exposure to the stressor and faster than what was expected based on previously published works. It can be assumed that dead cells from tissue damage were being packaged for expulsion. This occurred in all conditions except emersion in cold air, possibly indicating that the latter generates less immediate damage than the other acute conditions tested. Accordingly, Gianasi et al. (2016) suggested that keeping C. frondosa damp, outside water, at 4–5°C would yield the least severe tissue damage and highest survival rate during transport from the wharfs to the plants. Here, sea cucumbers emersed at cold temperature also showed the slowest cloacal openings rhythm (upon being immersed again), even below controls, suggesting they rapidly resumed a resting state. This trend is substantiated by the behavioral scores; i.e., individuals exposed to warm air temperature reached peak scores early during the experiment (indicative of stress), in contrast to individuals exposed to cold air, which maintained scores similar to baseline values.
As anticipated, the cellular immune responses in Cucumaria frondosa mirrored the increasing trend in cortisol levels recorded under the most severe stressors tested. While previous studies have described a cortisol increase in sea cucumbers (A. japonicus) exposed to stressors (Hou et al., 2019) or reported rises in coelomocytes and in cortisol under stress (Chen et al., 2018a,b; Hou et al., 2019), to our knowledge a link between the two factors has never been reported, although hormone research in vertebrates has shown that glucocorticoids (including cortisol) are the regulators of immune responses (i.e., increase in leukocytes and granulocytes; Ince et al., 2019). While the correlation between the rises in cortisol and in coelomocyte densities remains fragmentary in the present study, it highlights the need to tease out the link between the cellular and hormonal responses in Holothuroidea. Recently, Hou et al. (2019) determined that peak cortisol levels in A. japonicus were reached several hours into emersion and then slowly dropped toward baseline levels over the following 20 h. In C. frondosa the rise in cortisol could be necessary to generate a pool of free coelomocytes from their marginated (i.e., attached to the body wall) forms, as seen in Caulier et al. (2020). In line with this, cortisol increases were noticed in three of the four conditions tested, but emersion in warm air generated the most defined trend, which in combination with the other biomarkers (behavioral and cellular), points to this being the most detrimental treatment tested. In support, So et al. (2010) demonstrated that water temperatures above 18°C were deleterious for juveniles and adults of C. frondosa. Inversely, emersion in cold air coincided with a minimal cellular response, no measurable cortisol increase, and mild behavior scores, reinforcing that it is not an immediately threatening condition, at least for a short time, as suggested by Gianasi et al. (2016) in the study of transport methods.
Based on most markers measured after 23 h of recovery post exposure, it emerges that stressors may have long-lasting effects (i.e., beyond 24 h) on the wellbeing of sea cucumbers. Small aggregates showed an increase during recovery from cold air emersion and low salinity, and large aggregates multiplied during recovery from all conditions. There was no mortality in any of the treatments, but the presence of cell aggregates underlies the expulsion of materials resulting from infections or damaged tissues. Strangely, the stressors that elicited the mildest acute responses after 1 h (cold air and 22 psu salinity) yielded the highest counts of small and large aggregates during recovery, either due to a delayed response to the stress or to secondary infections. Inversely, the most severe acute responses (warm air and 15 psu) corresponded to the lowest aggregate counts during recovery, possibly because the immune response peaked earlier and had already begun to wane. It must be emphasized that while the stressors tested here reflected common harvesting and handling practices, the temporal scale is a conservative estimate of what sea cucumbers could endure over the preprocessing period, which may last 48 h – 1 week (Gianasi et al., 2016; S. Jobson, personal communication). Prolonged exposures to stress may lead to more severe damage and possibly more drastic immune responses, which may in turn translate into mortality and into economic loss (Wu et al., 2013; Qi et al., 2016).
Globally, the present study showed the potential of using multiple biometrics to characterize the immediate and long-term effects of stress on economically and ecologically important species like Cucumaria frondosa. Further studies might seek to refine the methodologies necessary to integrate the use of cortisol levels in the coelomic fluid as a rapid non-invasive biomarker of health in this and other species of invertebrates. Such a tool would greatly assist the design of sustainable harvesting, aquaculture and preprocessing protocols. Insights were also garnered from an ecological standpoint. While the optimal environmental conditions under which feeding, reproduction and development occur in C. frondosa are typically oceanic (Hamel and Mercier, 1996; So et al., 2010), this species has apparently developed notable capabilities to cope with harsh, even improbable, conditions in the short term. Such plasticity may explain its high biomasses and broad distribution range throughout a diversity of temperate and polar marine environments (Gianasi et al., 2020).
Data Availability Statement
The raw data supporting the conclusions of this article will be made available by the authors, without undue reservation.
Author Contributions
SJ, J-FH, and AM were responsible for conception of ideas, design, and writing of drafts. SJ and TH conducted the data collection and analysis. All authors contributed to the manuscript and approved the final version.
Funding
This research was partly funded by the Ocean Frontier Institute, through an award from the Canada First Research Excellence Fund to AM.
Conflict of Interest
The authors declare that the research was conducted in the absence of any commercial or financial relationships that could be construed as a potential conflict of interest.
Publisher’s Note
All claims expressed in this article are solely those of the authors and do not necessarily represent those of their affiliated organizations, or those of the publisher, the editors and the reviewers. Any product that may be evaluated in this article, or claim that may be made by its manufacturer, is not guaranteed or endorsed by the publisher.
Acknowledgments
We would like to thank the members of the Mercier lab for their technical and logistical support, with special thanks to Andrew Tucker for his help with data collection. We would like to acknowledge the invaluable expertise and support of Fabio Zanuzzo in designing a novel ELISA protocol and Kurt Gamperl for allowing use of his equipment to conduct the cortisol analysis. We would also like to thank Emaline Montgomery and Javier Santander for their contributions during the initial design of this study.
Supplementary Material
The Supplementary Material for this article can be found online at: https://www.frontiersin.org/articles/10.3389/fmars.2021.695753/full#supplementary-material
Footnotes
References
Ammendolia, J., Hamel, J.-F., and Mercier, A. (2018). Behavioural responses to hydrostatic pressure in selected echinoderms suggest hyperbaric constraint of bathymetric range. Mar. Biol. 165:145. doi: 10.1007/s00227-018-3399-7
Bang, F. B. (1975). A search in Asterias and Ascidia for the beginnings of vertebrate immune responses. Ann. N.Y. Acad. Sci. 266, 334–342. doi: 10.1111/j.1749-6632.1975.tb35115.x
Beck, G., and Habicht, G. S. (1996). Immunity and the invertebrates. Sci. Am. 275, 60–66. doi: 10.1038/scientificamerican1196-60
Binder, A. R. D., Pfaffl, M. W., Hiltwein, F., Geist, J., and Beggel, S. (2019). Does environmental stress affect cortisol biodistribution in freshwater mussels? Conserv. Physiol. 7:coz101. doi: 10.1093/conphys/coz101
Branco, P., Figueiredo, D., and da Silva, J. (2014). New insights into innate immune system of sea urchin: coelomocytes as biosensors for environmental stress. OA Biol. 18, 1–7.
Brothers, C. J., Harianto, J., McClintock, J. B., and Byrne, M. (2016). Sea urchins in a high CO2 world: the influence of acclimation on the immune response to ocean warming and acidification. Proc. R. Soc. B 283:20161501. doi: 10.1098/rspb.2016.1501
Byrne, M. (1986). The ultrastructure of the morula cells of Eupentacta quinquesemita (Echinodermata: Holothuroidea) and their role in the maintenance of the extracellular matrix. J. Morphol. 188, 179–189. doi: 10.1002/jmor.1051880205
Canicatti, C., D’Ancona, G., and Farina-Lipari, E. (1989). The Holothuria polii brown bodies. Ital. J. Zool. 56, 275–283. doi: 10.1080/11250008909355651
Canicatti, C., and Quaglia, A. (1991). Ultrastructure of Holothuria polii encapsulating body. J. Zool. 224, 419–429. doi: 10.1111/j.1469-7998.1991.tb06035.x
Canicatti, C., and Seymour, J. (1991). Evidence for phenoloxidase activity in Holothuria tubulosa (Echinodermata) brown bodies and cells. Parasitol. Res. 77, 50–53. doi: 10.1007/BF00934385
Caulier, G., Hamel, J.-F., and Mercier, A. (2020). From coelomocytes to colored aggregates: cellular components and processes involved in the immune response of the holothuroid Cucumaria frondosa. Biol. Bull. 239, 95–114. doi: 10.1086/710355
Chen, J., Ren, Y., Li, Y., and Xia, B. (2018a). Regulation of growth, intestinal microbiota, non-specific immune response and disease resistance of sea cucumber Apostichopus japonicus (Selenka) in biofloc systems. Fish Shellfish Immunol. 77, 175–186. doi: 10.1016/j.fsi.2018.03.053
Chen, J., Ren, Y., Wang, G., Xia, B., and Li, Y. (2018b). Dietary supplementation of biofloc influences growth performance, physiological stress, antioxidant status and immune response of juvenile sea cucumber Apostichopus japonicus (Selenka). Fish Shellfish Immunol. 72, 143–152. doi: 10.1016/j.fsi.2017.10.061
Chiaramonte, M., Inguglia, L., Vazzana, M., Deidun, A., and Arizza, V. (2019). Stress and immune response to bacterial LPS in the sea urchin Paracentrotus lividus (Lamarck, 1816). Fish Shellfish Immunol. 92, 384–394. doi: 10.1016/j.fsi.2019.06.017
Coteur, G., DeBecker, G., Warnau, M., Jangoux, M., and Dubois, P. (2002). Differentiation of immune cells challenged by bacteria in the common European starfish, Asterias rubens (Echinodermata). Eur. J. Cell Biol. 81, 413–418. doi: 10.1078/0171-9335-00254
D’Andrea-Winslow, L., Radke, D. W., Utecht, T., Kaneko, T., and Akasaka, K. (2012). Sea urchin coelomocyte arylsulfatase: a modulator of the echinoderm clotting pathway. Integr. Zool. 7, 61–73. doi: 10.1111/j.1749-4877.2011.00279.x
Dan-Sohkawa, M., Morimoto, M., and Kaneko, H. (1995a). In vitro reactions of coelomocytes against sheep red blood cells in the solitary ascidian Halocynthia roretzi. Zool. Sci. 12, 411–417. doi: 10.2108/zsj.12.411
Dan-Sohkawa, M., Morimoto, M., Mishima, H., and Kaneko, H. (1995b). Characterization of coelomocytes of the ascidian Halocynthia roretzi based on phase-contrast, time-lapse video and scanning electron microscopic observations. Zool. Sci. 12, 289–301. doi: 10.2108/zsj.12.289
de Freitas Rebelo, M., de Souza Figueiredo, E., Mariante, R. M., Nóbrega, A., de Barros, C. M., and Allodi, S. (2013). New insights from the oyster Crassostrea rhizophorae on bivalve circulating hemocytes. PLoS One 8:e57384. doi: 10.1371/journal.pone.0057384
Dushoff, J., Kain, M. P., and Bolker, B. M. (2019). I can see clearly now: reinterpreting statistical significance. Methods Ecol. Evol. 10, 756–759. doi: 10.1111/2041-210X.13159
Edds, K. T. (1980). The formation and elongation of filopodia during transformation of sea urchin coelomocytes. Cell Motil. 1, 131–140. doi: 10.1002/cm.970010110
Eliseikina, M. G., and Magarlamov, T. Y. (2002). Coelomocyte morphology in the holothurians Apostichopus japonicus (Aspidochirota: Stichopodidae) and Cucumaria japonica (Dendrochirota: Cucumariidae). Russ. J. Mar. Biol. 28:6. doi: 10.1023/A:1016801521216
Fontaine, A. R., and Lambert, P. (1977). The fine structure of the leucocytes of the holothurian, Cucumaria miniata. Can. J. Zool. 55, 1530–1544. doi: 10.1139/z77-198
Franchi, N., and Ballarin, L. (2017). Immunity in protochordates: the tunicate perspective. Front. Immunol. 8:674. doi: 10.3389/fimmu.2017.00674
Galimany, E., Baeta, M., and Ramón, M. (2018). Immune response of the sea cucumber Parastichopus regalis to different temperatures: implications for aquaculture purposes. Aquaculture 497, 357–363. doi: 10.1016/j.aquaculture.2018.08.005
Gianasi, B. L., Hamel, J.-F., and Mercier, A. (2016). Experimental test of optimal holding conditions for live transport of temperate sea cucumbers. Fish. Res. 174, 298–308. doi: 10.1016/j.fishres.2015.11.004
Gianasi, B. L., Hamel, J.-F., Montgomery, E. M., Sun, J., and Mercier, A. (2020). Current knowledge on the biology, ecology, and commercial exploitation of the sea cucumber Cucumaria frondosa. Rev. Fish. Sci. Aquac. doi: 10.1080/23308249.2020.1839015
Gianasi, B. L., Verkaik, K., Hamel, J.-F., and Mercier, A. (2015). Novel use of pit tags in sea cucumbers: promising results with the commercial species Cucumaria frondosa. PLoS One 10:e0127884. doi: 10.1371/journal.pone.0127884
Gorshkov, A. N., Blinova, M. I., and Pinaev, G. P. (2009). Ultrastructure of coelomic epithelium and coelomocytes of the starfish Asterias rubens L. in norm and after wounding. Cell Tiss. Biol. 3, 477–490. doi: 10.1134/S1990519X09050113
Grantham, B. A., Eckert, G. L., and Shanks, A. L. (2003). Dispersal potential of marine invertebrates in diverse habitats. Ecol. Appl. 13, 108–116. doi: 10.1890/1051-0761(2003)013[0108:dpomii]2.0.co;2
Gross, P. S., Al-Sharif, W. Z., Clow, L. A., and Smith, L. C. (1999). Echinoderm immunity and the evolution of the complement system. Dev. Comp. Immunol. 23, 429–442. doi: 10.1016/S0145-305X(99)00022-1
Hamel, J.-F., Jobson, S., Caulier, G., and Mercier, A. (2021). Evidence of anticipatory immune and hormonal responses to predation risk in an echinoderm. Sci. Rep. 11:10691. doi: 10.1038/s41598-021-89805-0
Hamel, J.-F., and Mercier, A. (1996). Early development, settlement, growth, and spatial distribution of the sea cucumber Cucumaria frondosa (Echinodermata: Holothuroidea). Can. J. Fish. Aquat. Sci. 53, 253–271. doi: 10.1139/f95-186
Hamel, J.-F., Sun, J., Gianasi, B. L., Montgomery, E. M., Kenchington, E. L., Burel, B., et al. (2019). Active buoyancy adjustment increases dispersal potential in benthic marine animals. J. Anim. Ecol. 88, 820–832. doi: 10.1111/1365-2656.12943
Hetzel, H. R. (1965). Studies on holothurian coelomocytes. ii. The origin of coelomocytes and the formation of brown bodies. Biol. Bull. 128, 102–111. doi: 10.2307/1539393
Hossain, A., Dave, D., and Shahidi, F. (2020). Northern sea cucumber (Cucumaria frondosa): a potential candidate for functional food, nutraceutical, and pharmaceutical sector. Mar. Drugs 18:274. doi: 10.3390/md18050274
Hou, S., Jin, Z., Jiang, W., Chi, L., Xia, B., and Chen, J. (2019). Physiological and immunological responses of sea cucumber Apostichopus japonicus during desiccation and subsequent resubmersion. PeerJ 7:e7427. doi: 10.7717/peerj.7427
Huo, D., Sun, L., Ru, X., Zhang, L., Lin, C., Liu, S., et al. (2018). Impact of hypoxia stress on the physiological responses of sea cucumber Apostichopus japonicus: respiration, digestion, immunity and oxidative damage. PeerJ 6:e4651. doi: 10.7717/peerj.4651
Huo, D., Sun, L., Zhang, L., Ru, X., Liu, S., and Yang, H. (2019). Metabolome responses of the sea cucumber Apostichopus japonicus to multiple environmental stresses: heat and hypoxia. Mar. Pollut. Bull. 138, 407–420. doi: 10.1016/j.marpolbul.2018.11.063
Ince, L. M., Weber, J., and Scheiermann, C. (2019). Control of leukocyte trafficking by stress-associated hormones. Front. Immunol. 9:3143. doi: 10.3389/fimmu.2018.03143
Jans, D., Dubois, P., and Jangoux, M. (1995). Defensive mechanisms of holothuroids (Echinodermata): formation, role, and fate of intracoelomic brown bodies in the sea cucumber Holothuria tubulosa. Cell Tissue Res. 283, 99–106. doi: 10.1007/s004410050517
Kindred, J. E. (1924). The cellular elements in the perivisceral fluid of echinoderms. Biol. Bull. 46, 228–251. doi: 10.2307/1536725
Li, Q., Qi, R., Wang, Y., Ye, S., Qiao, G., and Li, H. (2013). Comparison of cells free in coelomic and water-vascular system of sea cucumber, Apostichopus japonicus. Fish Shellfish Immunol. 35, 1654–1657. doi: 10.1016/j.fsi.2013.07.020
Li, Q., Ren, Y., Luan, L., Zhang, J., Qiao, G., Wang, Y., et al. (2019). Localization and characterization of hematopoietic tissues in adult sea cucumber, Apostichopus japonicus. Fish Shellfish Immunol. 84, 1–7. doi: 10.1016/j.fsi.2018.09.058
Majeske, A. J., Bayne, C. J., and Smith, L. C. (2013). Aggregation of sea urchin phagocytes is augmented in vitro by lipopolysaccharide. PLoS One 8:e61419. doi: 10.1371/journal.pone.0061419
Melillo, D., Marino, R., Italiani, P., and Boraschi, D. (2018). Innate immune memory in invertebrate metazoans: a critical appraisal. Front. Immunol. 9:1915. doi: 10.3389/fimmu.2018.01915
Mercier, A., Battaglene, S. C., and Hamel, J.-F. (1999). Daily burrowing cycle and feeding activity of juvenile sea cucumbers Holothuria scabra in response to environmental factors. J. Exp. Mar. Biol. Ecol. 239, 125–156. doi: 10.1016/S0022-0981(99)00034-9
Nelson, E. J., MacDonald, B. A., and Robinson, S. M. C. (2012). The absorption efficiency of the suspension-feeding sea cucumber, Cucumaria frondosa, and its potential as an extractive integrated multi-trophic aquaculture (IMTA) species. Aquaculture 370, 19–25. doi: 10.1016/j.aquaculture.2012.09.029
Pei, S., Dong, S., Wang, F., Tian, X., and Gao, Q. (2012). Effects of density on variation in individual growth and differentiation in endocrine response of Japanese sea cucumber Apostichopus japonicus (Selenka). Aquaculture 356, 398–403. doi: 10.1016/j.aquaculture.2012.04.032
Pinsino, A., Thorndyke, M. C., and Matranga, V. (2007). Coelomocytes and post-traumatic response in the common sea star Asterias rubens. Cell Stress Chaperones 12:331. doi: 10.1379/CSC-288.1
Purcell, S. W. (2014). Value, market preferences and trade of beche-de-mer from Pacific island sea cucumbers. PLoS One 9, 1–8. doi: 10.1371/journal.pone.0095075
Purcell, S. W., Mercier, A., Conand, C., Hamel, J.-F., Toral-Granda, M. V., Lovatelli, A., et al. (2013). Sea cucumber fisheries: global analysis of stocks, management measures and drivers of overfishing: management of sea cucumber fisheries. Fish Fish. 14, 34–59. doi: 10.1111/j.1467-2979.2011.00443.x
Qi, H., Fu, H., Dong, X., Feng, D., Li, N., Wen, C., et al. (2016). Apoptosis induction is involved in UVA-induced autolysis in sea cucumber Stichopus japonicus. J. Photoch. Photobio. B. 158, 130–135. doi: 10.1016/j.jphotobiol.2016.02.034
Ramírez-Gómez, F., Aponte-Rivera, F., Méndez-Castaner, L., and García-Arrarás, J. E. (2010). Changes in holothurian coelomocyte populations following immune stimulation with different molecular patterns. Fish Shellfish Immunol. 29, 175–185. doi: 10.1016/j.fsi.2010.03.013
Ridder, C., and Jangoux, M. (1984). Intracoelomic parasitic: sporozoa in the burrowing spatangoid echinoid Echinocardium cordatum: coelomocyte reaction and formation of brown bodies. Helgolander Meeresunters 37, 225–231. doi: 10.1007/BF01989307
Rinkevich, B., and Müller, W. E. G. (1996). Invertebrate Immunology, Vol. 15. Berlin: Spring Sci Business Media.
Russell, M. P. (2013). Echinoderm responses to variation in salinity. Adv. Mar. Biol. 66, 171–212. doi: 10.1016/B978-0-12-408096-6.00003-1
San Miguel-Ruiz, J. E., and García-Arrarás, J. E. (2007). Common cellular events occur during wound healing and organ regeneration in the sea cucumber Holothuria glaberrima. BMC Dev. Biol. 7:1–19. doi: 10.1186/1471-213X-7-115
Sandner, M., Lois, G., Streit, F., Zeier, P., Kirsch, P., Wüst, S., et al. (2020). Investigating individual stress reactivity: high hair cortisol predicts lower acute stress responses. Psychoneuroendocrino 118:104660. doi: 10.1016/j.psyneuen.2020.104660
Satyavathi, V. V., Minz, A., and Nagaraju, J. (2014). Nodulation: an unexplored cellular defense mechanism in insects. Cell Signal. 26, 1753–1763. doi: 10.1016/j.cellsig.2014.02.024
Smith, L. C., Arizza, V., Barela Hudgell, M. A., Barone, G., Bodnar, A. G., Buckley, K. M., et al. (2018). “Echinodermata: the complex immune system in echinoderms,” in Advances in Comparative Immunology, ed. E. Cooper (Cham: Springer), 409–501.
Smith, L. C., and Davidson, E. H. (1992). The echinoid immune system and the phylogenetic occurrence of immune mechanisms in deuterostomes. Immunol. Today. 13, 356–362. doi: 10.1016/0167-5699(92)90172-4
Smith, L. C., and Davidson, E. H. (1994). The echinoderm immune system: characters shared with vertebrate immune systems and characters arising later in deuterostome phylogeny. Ann. NY Acad. Sci. 712, 213–226. doi: 10.1111/j.1749-6632.1994.tb33575.x
So, J. J., Hamel, J.-F., and Mercier, A. (2010). Habitat utilisation, growth and predation of Cucumaria frondosa: implications for an emerging sea cucumber fishery. Fish. Manag. Ecol. 17, 473–484. doi: 10.1111/j.1365-2400.2010.00747.x
Söderhäll, K. (ed.) (2010). Invertebrate Immunity. New York, N.Y: Springer Science + Business Media.
Sparks, A. K. (1976). Inflammation and wound repair in oysters. Mar. Fish. Rev. 38, 2–4. doi: 10.1111/j.1524-475x.2011.00754.x
Suh, S. S., Hwang, J., Park, M., Park, S. Y., Ryu, T. K., Lee, S., et al. (2014). Hypoxia-modulated gene expression profiling in sea urchin (Strongylocentrotus nudus) immune cells. Ecotox. Environ. Safe. 109, 63–69. doi: 10.1016/j.ecoenv.2014.08.011
Sun, J., Hamel, J.-F., Gianasi, B. L., Graham, M., and Mercier, A. (2020). Growth, health and biochemical composition of the sea cucumber Cucumaria frondosa after multi-year holding in effluent waters of land-based salmon culture. Aquacult. Environ. Interact. 12, 139–151. doi: 10.3354/aei00356
Sun, J., Hamel, J.-F., and Mercier, A. (2018). Influence of flow on locomotion, feeding behaviour and spatial distribution of a suspension-feeding sea cucumber. J. Exp. Biol. 221, jeb189597. doi: 10.1242/jeb.189597
Sun, L., Chen, M., Yang, H., Wang, T., Liu, B., Shu, C., et al. (2012). Large scale gene expression profiling during intestine and body wall regeneration in the sea cucumber Apostichopus japonicus. Comp. Biochem. Phys. D Genomics Proteomics 6, 195–205. doi: 10.1016/j.cbd.2011.03.002
Toral-Granda, V., Lovatelli, A., and Vasconcellos, M. (2008). Sea cucumbers: a global review of fisheries and trade. FOA Fish. Tech. Pap. 516:317. doi: 10.1111/j.1467-2979.2010.00397.x
Uren Webster, T. M., Rodriguez-Barreto, D., Consuegra, S., and Garcia de Leaniz, C. (2020). Cortisol-related signatures of stress in the fish microbiome. Front. Microbiol. 11:1621. doi: 10.3389/fmicb.2020.01621
Wang, F., Yang, H., Gao, F., and Liu, G. (2008). Effects of acute temperature or salinity stress on the immune response in sea cucumber, Apostichopus japonicus. Comp. Biochem. Phys. A Mol. Integr. Physiol. 151, 491–498. doi: 10.1016/j.cbpa.2008.06.024
Wasserstein, R. L., and Lazar, N. A. (2016). The ASA statement on p-values: context, process, and purpose. Am. Stat. 70, 129–133. doi: 10.1080/00031305.2016.1154108
Wasserstein, R. L., Schirm, A. L., and Lazar, N. A. (2019). Moving to a world beyond “p< 0.05”. Am. Stat. 73(sup 1), 1–19. doi: 10.1080/00031305.2019.1583913
Wu, H. T., Li, D. M., Zhu, B. W., Sun, J. J., Zheng, J., Wang, F. L., et al. (2013). Proteolysis of noncollagenous proteins in sea cucumber, Stichopus japonicus, body wall: characterisation and the effects of cysteine protease inhibitors. Food Chem. 141, 1287–1294. doi: 10.1016/j.foodchem.2013.03.088
Xia, B., Ren, Y., Wang, J., Sun, Y., and Zhang, Z. (2017). Effects of feeding frequency and density on growth, energy budget and physiological performance of sea cucumber Apostichopus japonicus (Selenka). Aquaculture 466, 26–32. doi: 10.1016/j.aquaculture.2016.09.039
Xing, K., Yang, H., and Chen, M. (2008). Morphological and ultrastructural characterization of the coelomocytes in Apostichopus japonicus. Aquat. Biol. 2, 85–92. doi: 10.3354/ab00038
Xu, B., Lang, L., Li, S. Z., Guo, J. R., Wang, J. F., Wang, D., et al. (2019). Cortisol excess-mediated mitochondrial damage induced hippocampal neuronal apoptosis in mice following cold exposure. Cells 8:612. doi: 10.3390/cells8060612
Xue, Z., Li, H., Wang, X., Li, X., Liu, Y., Sun, J., et al. (2015). A review of the immune molecules in the sea cucumber. Fish Shellfish Immunol. 44, 1–11. doi: 10.1016/j.fsi.2015.01.026
Yang, H., Hamel, J.-F., and Mercier, A. (2015). The Sea Cucumber Apostichopus japonicus: History, Biology and Aquaculture. Cambridge, MA: Academic Press.
Yoccoz, N. G. (1991). Use, overuse, and misuse of significance tests in evolutionary biology and ecology. Bull. Ecol. Soc. Am. 72, 106–111.
Young, C. M., and Chia, F. S. (1982). Factors controlling spatial distribution of the sea cucumber Psolus chitonoides: settling and post-settling behavior. Mar. Biol. 69, 195–205. doi: 10.1007/BF00396899
Keywords: echinoderm, aquaculture, coelomocytes, cortisol, stress, salinity, temperature, sea cucumber
Citation: Jobson S, Hamel J-F, Hughes T and Mercier A (2021) Cellular, Hormonal, and Behavioral Responses of the Holothuroid Cucumaria frondosa to Environmental Stressors. Front. Mar. Sci. 8:695753. doi: 10.3389/fmars.2021.695753
Received: 15 April 2021; Accepted: 13 August 2021;
Published: 01 September 2021.
Edited by:
Judith Meyer, GEOMAR Helmholtz Centre for Ocean Research Kiel, GermanyReviewed by:
Jun Ding, Dalian Ocean University, ChinaSau Pinn Woo, Universiti Sains Malaysia, Malaysia
Vinicius Queiroz, University of São Paulo, Brazil
Copyright © 2021 Jobson, Hamel, Hughes and Mercier. This is an open-access article distributed under the terms of the Creative Commons Attribution License (CC BY). The use, distribution or reproduction in other forums is permitted, provided the original author(s) and the copyright owner(s) are credited and that the original publication in this journal is cited, in accordance with accepted academic practice. No use, distribution or reproduction is permitted which does not comply with these terms.
*Correspondence: Sara Jobson, smjobson@mun.ca